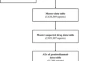
Overview
DARU Journal of Pharmaceutical Sciences is an international journal publishing significant studies from basic research to clinical investigations.
- Committed to publishing original articles, systematic reviews, meta-analyses, general reviews, mini-reviews, short communications, and editorials.
- Considers a wide range of submissions from the global scientific community.
- Indexed in Medline, Scopus, PubMed, and Science Citation Index.
- Editor-in-Chief
-
- Mohammad Abdollahi
- Impact factor
- 3.6 (2022)
- 5 year impact factor
- 4.3 (2022)
- Submission to first decision (median)
- 24 days
- Downloads
- 220,960 (2023)
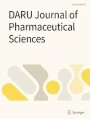
Latest articles
Journal updates
Journal information
- Electronic ISSN
- 2008-2231
- Abstracted and indexed in
-
- BIOSIS
- Baidu
- CAB Abstracts
- CLOCKSS
- CNKI
- CNPIEC
- Chemical Abstracts Service (CAS)
- Dimensions
- EBSCO
- EMBASE
- Gale
- Google Scholar
- Japanese Science and Technology Agency (JST)
- Medline
- Meta
- Naver
- OCLC WorldCat Discovery Service
- Portico
- ProQuest
- PubMedCentral
- Reaxys
- SCImago
- SCOPUS
- Science Citation Index Expanded (SCIE)
- TD Net Discovery Service
- UGC-CARE List (India)
- Wanfang
- Copyright information